Not only does evaporation drive global weather systems and reduce ambient dry-bulb temperatures, but its significant sensible cooling power has also been employed by humans for thousands of years to improve comfort. Evaporating water can provide a ‘free’ – or comparatively low-cost – source of cooling for buildings. This article will consider the evaporative cooling process as used in building environmental systems.
CPD refresher
To get the most benefit from this article, a basic understanding of psychrometry is required. For a refresher on the application of the psychrometric chart and the associated air processes, see CIBSE Journal CPD modules 3, 7 and 9.
The vaporisation of water requires a significant amount of energy, whether it is for the conversion to steam in a boiling process or the more gradual evaporation from the surface of cooler water. If the water’s vapour pressure is higher than the surrounding vapour pressure, some water molecules will escape as vapour from the water’s surface into the adjacent space. As the vapour pressure builds in the adjacent space (with the rising number of water molecules), increased numbers of molecules will also re-enter the water surface. If the vapour pressure in the adjacent space reaches the same value as that of the liquid water, an equilibrium will be reached, with no net evaporation from the water’s surface. At this point, the surrounding space – normally air – is said to be saturated with water vapour and it will have reached its ‘dew point’. If the temperature of the water is then increased, its energy and vapour pressure will rise, so promoting more evaporation from the liquid and moving more latent energy into the surrounding space. Evaporation will accelerate if the temperature of the surrounding unsaturated air climbs.
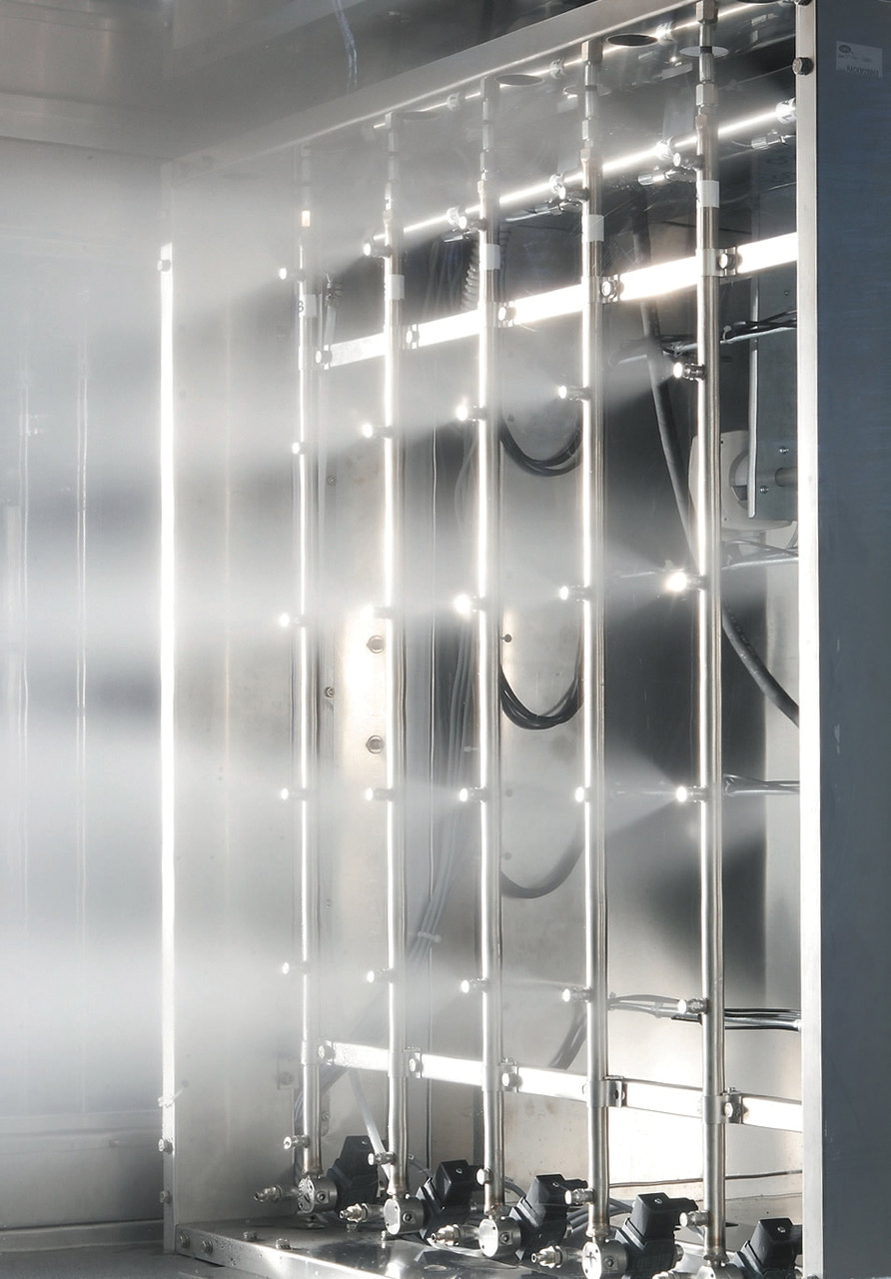
Figure 1: An atomising spray humidifier or ‘air washer’ (Source: CIBSE KS19)
The energy required to evaporate water is dependent on the water temperature. As a body of water increases in temperature, the ‘latent heat of evaporation’ will reduce slightly, as the internal energy of the molecules rises with increased temperature. The latent heat of evaporation is typically taken as 2,450kJ/kg at temperatures used in building environmental applications. This compares with a water specific heat capacity of only 4.18kJ/kgK – the heat needed to increase 1kg of liquid water by 1K. Evaporation reduces the total energy in the remaining water, so potentially cooling it. If the temperature of the adjacent air is higher than that of the water, it will supply heat to the water that will, in turn, maintain or increase the water evaporation rate. The primary driving force is the difference in vapour pressure and it is the availability of heat – from the air or the water itself – that powers the transfer.
The rate of natural evaporation from the water’s still surface into motionless air will be relatively slow. A thin layer above the water will soon become saturated with water vapour, and then further evaporation will rely on that water vapour, adjacent to the surface, diffusing into the larger space. However, if the air is moved – for example, blown with a fan across the surface or the water is agitated or moved – then the rate of evaporation will increase substantially. The actual rates of evaporation are complex to evaluate. There are empirical formulae that have been developed – many associated with the evaporative losses from swimming pools. (For a more technical, in-depth discussion of evaporation rates, see the paper by Mirza Mohammed Shah Methods for Calculation of Evaporation from Swimming Pools and Other Water Surfaces.1)
When water is sprayed into the air by, for example, the atomising spray in Figure 1, the multiple tiny droplets create a large combined area for evaporation and heat flow. The actual relationship of heat and mass transfer is again complex. The paper by Da Silva and others, Building Thermal Performance Simulation with Direct Evaporative Cooling by Water Spray Vaporization2 provides some good background.
Assuming that there is no heat added to the water spray to power the evaporation – apart from that coming from the air and the water itself – then this is known as an ‘adiabatic’ process. The net vaporisation will continue as long as the air has a vapour pressure below that of the water, as shown in the psychrometric chart in Figure 2. As the vapour pressure rises, there will be an increase in the air’s moisture content,
g (kg/kgda) – also known as ‘humidity ratio’ or ‘specific humidity’.
The dry-bulb temperature of the air will reduce – compared with when the spray was initially turned on – as the sensible heat, both from the air and from the spray water, is used to provide energy, as the water molecules vaporise from the droplets. As the air moisture content rises from gA to gB, the dry-bulb temperature falls from θA to θB, so enabling ‘evaporative cooling’. It is unlikely that the air will reach a fully saturated state at X. The effectiveness (or efficiency) of the humidification process is given by the ratio of
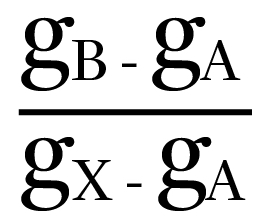
and is affected by the velocity of the air flow, the aerosol size, and the effective wetted area, as well as the air and water temperatures and vapour pressures.
The thermodynamic characteristics of water vapour by virtue of it having a near unity value of ‘Lewis’ number3, are such that at the point of saturation – when the air dry-bulb temperature is at the adiabatic saturation temperature – the thermodynamic wet-bulb temperature will equal the dry-bulb temperature. At this point, the air is said to have reached its ‘dew point’. If the air dry-bulb temperature was subsequently to be reduced, condensation will occur as the molecules of water vapour return to liquid as the saturated vapour pressure is reduced.
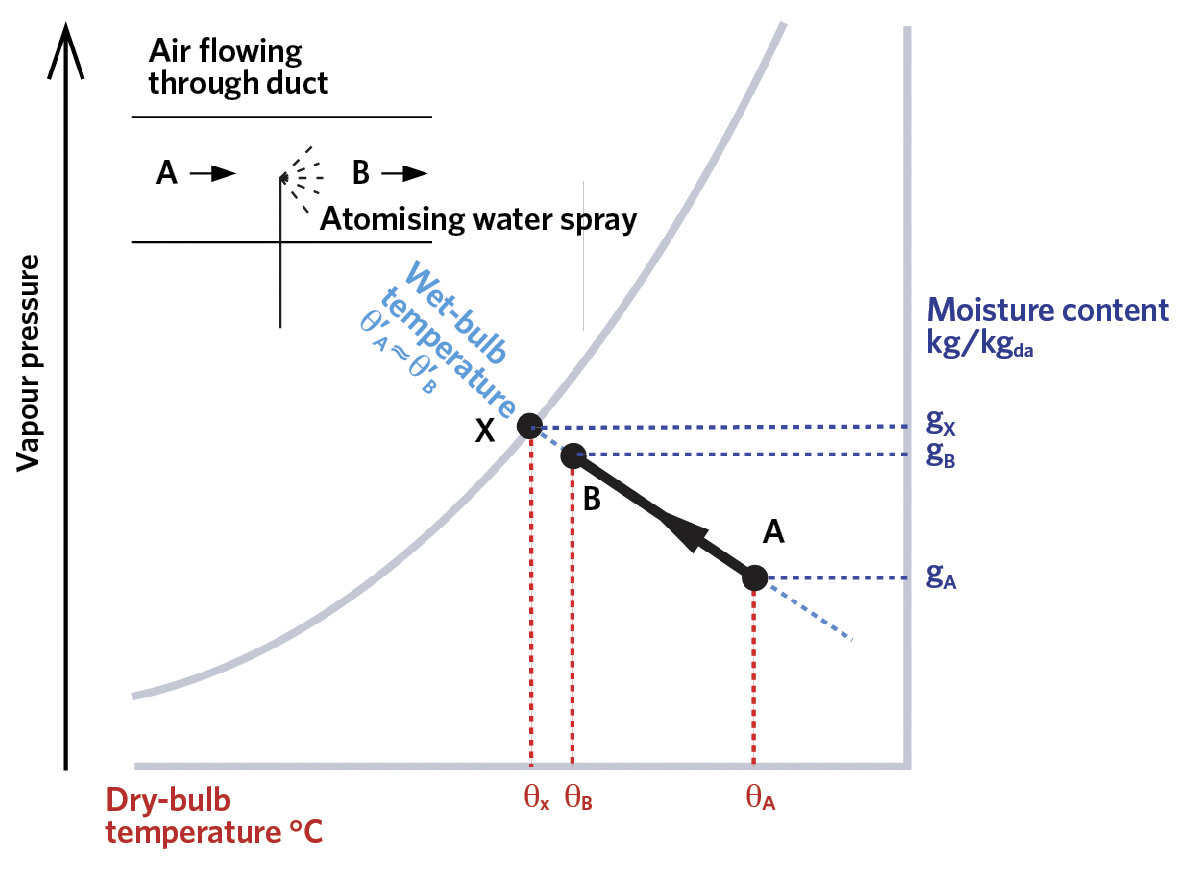
Figure 2: The adiabatic cooling process
The practical wet-bulb temperature, θ’ (°C), may be measured by a psychrometer – such as the sling psychrometer in Figure 3 – and although it is unlikely to coincide exactly with the value of the thermodynamic wet-bulb temperature, it is considered sufficiently accurate for practical air conditioning assessment and control. As defined by Roger Legg4, the measured wet-bulb temperature is simply and practically ‘the temperature obtained with a thermometer whose bulb is covered with a muslin sleeve, which is kept moist with distilled/clean water, freely exposed to the air and free from radiation’. The energy required for the evaporation of the water from the wetted wick into the surrounding air (at a dry-bulb temperature θ°C) is mainly taken from the thermometer’s bulb, so reducing the temperature indicated by the wet-bulb thermometer. The difference in the values of the wet-bulb temperature, compared with that of the air’s dry-bulb temperature, (θ – θ’) (K), is known as the ‘wet-bulb depression’.
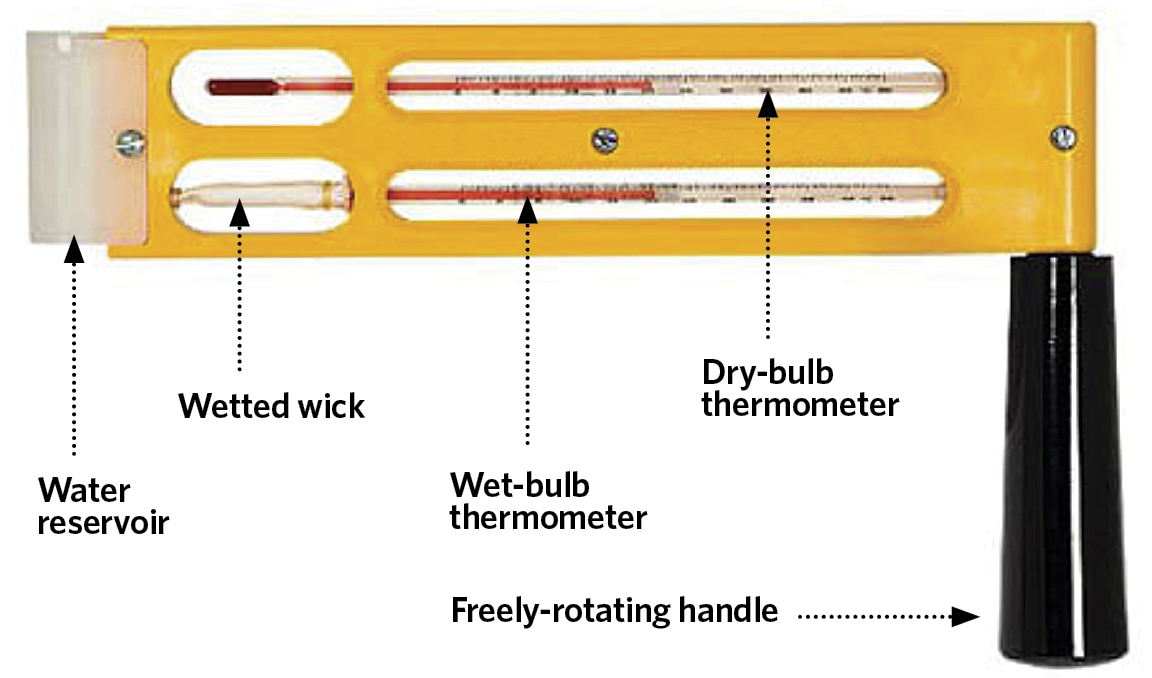
Figure 3: Sling psychrometer
The wet-bulb temperature is useful as a means of determining the moisture content and specific enthalpy of the air, using a psychrometric chart or equation; these can then be applied to calculations, including those to determine humidification requirements and evaporative cooling potential. The air’s wet-bulb temperature effectively sets the lowest dry-bulb temperature that can be approached when using evaporative cooling. So, as an example, considering external summer temperatures in London5, the wet-bulb temperature rarely (0.04% of hours) exceeds 22°C, whereas the dry-bulb temperature exceeds 22°C for more than 13% of the time. In this specific example, evaporative cooling could potentially be applied for at least 400 of the warmest hours – that is, 13% of the hours – of the summer cooling period to produce air approaching a dry-bulb temperature of 22°C.
This can significantly reduce the required refrigeration peak capacity used for comfort cooling, as well as cut energy consumption. Any potential energy savings – or reduction in installed plant capacity – will be dependent on location and application. In more arid climates, the opportunity to use evaporative cooling is greater but will, of course, depend on the availability of suitable water. Appropriate modelling and simulation are required to make a total life-cycle assessment of predicted performance, which should include the cost and availability of water.
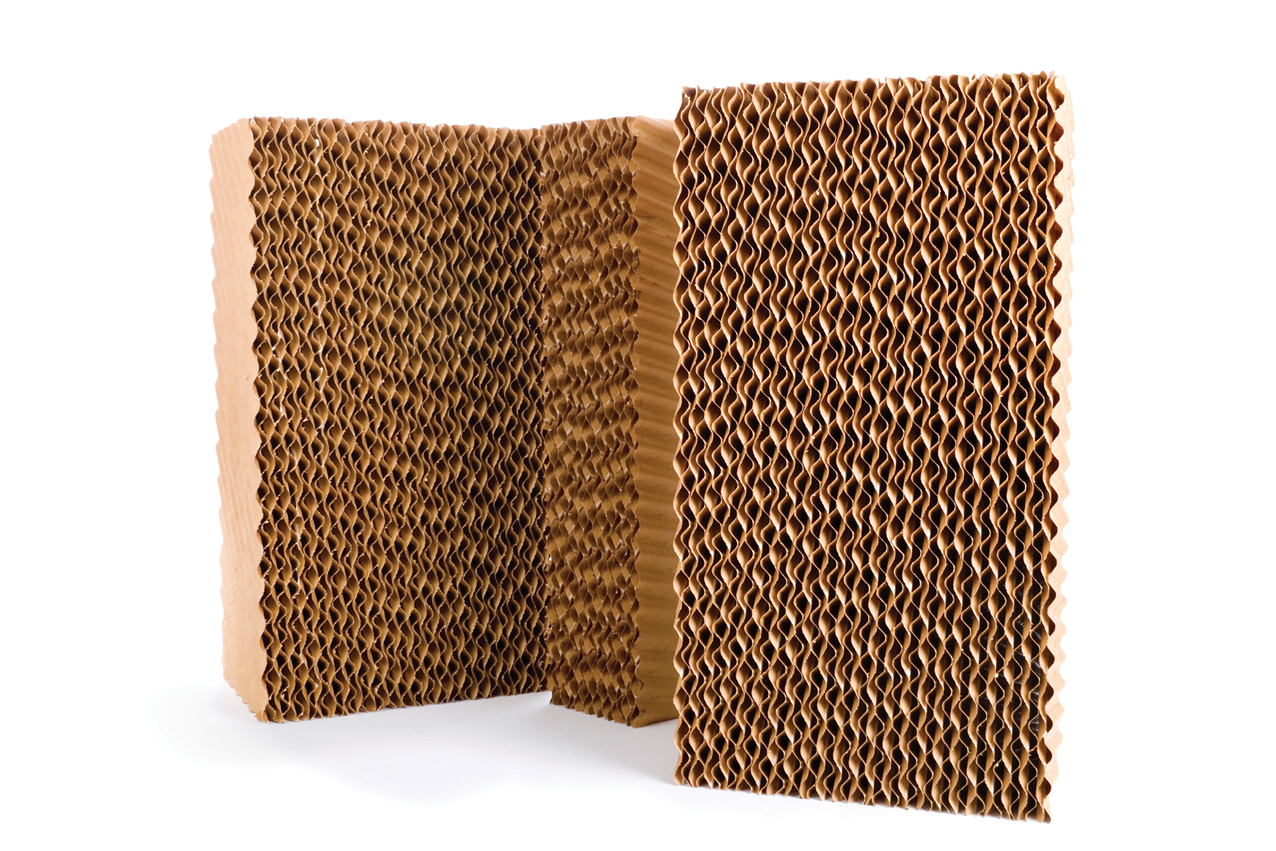
Figure 4: Evaporative cooling pad
If water that is sprayed into an unsaturated airstream has a higher temperature than the air dry-bulb temperature, it can both heat and humidify the air. Spray water that has a temperature below the air dry-bulb temperature but above the air dew point temperature will produce humidification and evaporative cooling. And if the water is introduced below the dew point temperature of the air, this could both cool and dehumidify the air as the water vapour in the air condenses into the cool sprayed water. (This is discussed in more detail in Legg4, Chapter 2.)
To increase the exposed water surface area, evaporative cooling pads – such as the material shown in Figure 4 – can be used, where water is supplied through a manifold at the top of the pad and allowed to trickle down as air flows through the matrix. A traditional alternative to this was to spray the water over the (inactive) chilled water coils in the air-handling unit. Known as a ‘spray coil’, this process provides an increased wetted surface area compared with using a simple spray. The spray systems available today produce atomised water that can be closely controlled to ensure effective humidification, often without using an extended wetted surface.
It should be noted that the use of any recirculated water – as would have traditionally been the case in a spray coil – must be carefully considered, as doing so can create a significant opportunity for the accumulation of bacteria. The majority of evaporative coolers will use fresh water, so will present a much-reduced risk. Both freshwater and recirculated systems will also ‘wash’ particulate matter from the airstream, adding to the risk of organic matter accumulation. The July 2000 ASHRAE Journal article ‘Operation and maintenance of evaporative coolers’ offers a useful source of suitable operational practice.
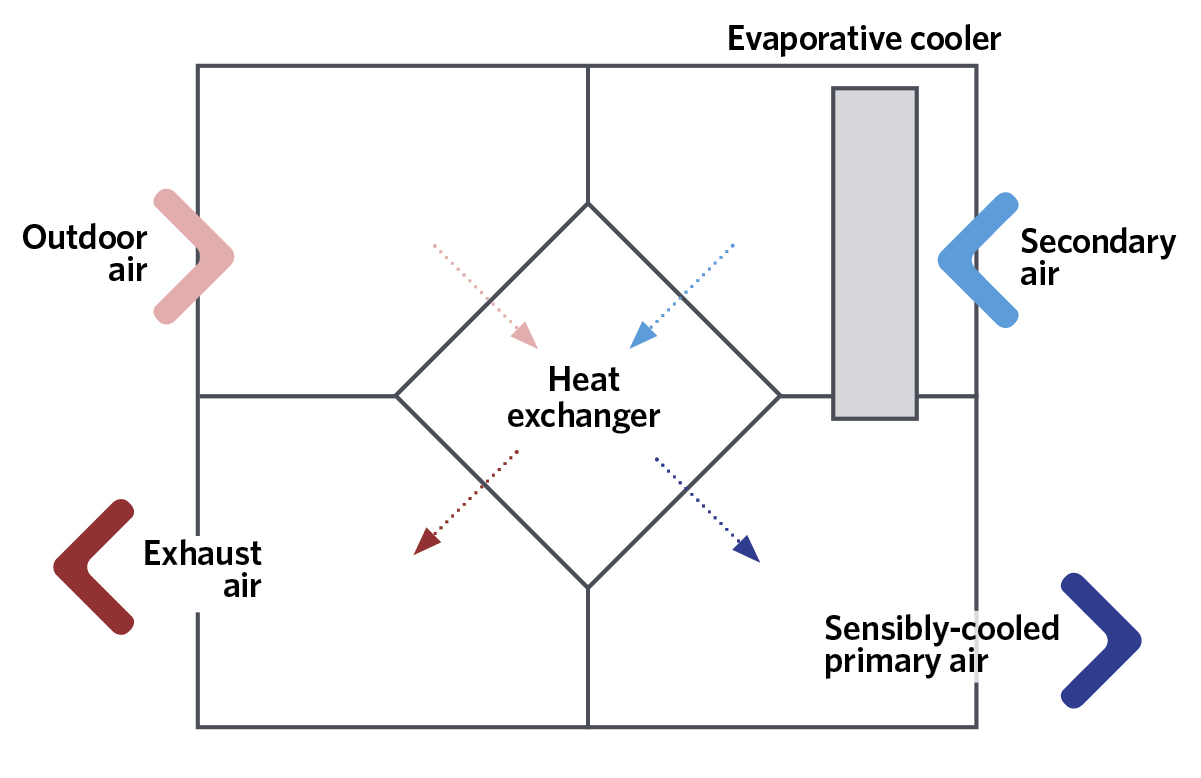
Figure 5: Schematic of a simple example of indirect evaporative cooling
By its very nature, direct evaporative cooling will increase the air moisture content. However, in many cases, this may not be desired.
The increase in moisture content can be mitigated in some applications either by mixing the humidified and cooled air with outdoor air that has bypassed the evaporative cooler – or by simply using recirculated room air. Another increasingly popular method is to use indirect evaporative cooling, as shown in the simplified example in Figure 5. The secondary airstream could be the return air from the conditioned space or outdoor air. The secondary air, which has been evaporatively cooled, will – through the heat exchanger – pre-cool the incoming outdoor air to reduce its dry-bulb temperature, while not directly affecting its moisture content, so also reducing its adiabatic saturation and wet-bulb temperatures. There are various arrangements that are employed to increase the potential cooling effect. For example, direct evaporative cooling could be applied to the already sensibly-cooled primary air to reduce further the dry-bulb temperature while, of course, raising its moisture content.
If evaporative cooling was to be used as the sole means of cooling in a building system, there is likely to be an increased supply air volume flow rate, compared with that when using mechanical refrigeration cooling, as supply temperatures may be higher. Evaporative cooling is often used in conjunction with a conventional refrigeration system, or together with desiccant dehumidification. This combination can provide lower supply air temperatures, as well as being able to cope with room latent cooling loads.
© Tim Dwyer, 2017.
Further reading:
- One of the most comprehensive books that considers the theory and practice that underpin evaporative cooling in building applications is Air humidification, available through Carel e-book-request.
- There is some coverage in CIBSE Guide B3 2016, section 3.2.4.7 Humidifiers and section 3.3.3.9 Evaporative cooling. The CIBSE Knowledge Series KS19 Humidification provides a more extensive background, and ASHRAE Applications 2015 Chapter 52 Evaporative cooling offers some useful commentary of specific applications.
References:
- Shah, M M, Methods for Calculation of Evaporation from Swimming Pools and Other Water Surfaces, ASHRAE Transactions, Volume 120, Part 2, 2014.
- Da Silva, A C S B et al, Building Thermal Performance Simulation with Direct Evaporative Cooling by Water Spray Vaporization, HVAC&R Research, Volume 12(3), pp669-692, July 2006.
- Jones, W P, Air Conditioning Engineering, Section 3, Routledge, 2000.
- Legg, R C, Air Conditioning Systems: Design and Integration, Chapter 1, Batsford, 1991.
- CIBSE Guide A 2015, table A2.9g.